Cell & gene therapies and the evolving role of personalized medicine
Cell Gene Therapy Insights 2016; 2(2), 277-286.
10.18609/cgti.2016.034
Personalized medicine is an innovative approach to disease prevention and treatment that takes into account differences in how individuals respond to medication. Advances in personalized medicine have already led to powerful new discoveries and approved treatments that are tailored to specific characteristics of individuals, such as a person’s genetic makeup, or the genetic profile of an individual’s tumor. Autologous cell therapies are arguably one of the most personalized forms of medicine, using a patient’s own cells to generate a bespoke product that is only administered back to the original donor. This article discusses the emerging role of personalized medicine in various cell and gene therapies, its role in health economics and challenges to its implementation.
The term personalized medicine is often considered as providing “the right patient with the right therapy at the right dose at the right time”. It encompasses approaches such as “precision medicine”, “stratified medicine” and “pharmacogenomics” Figure 1, and is an alternative to the traditional process for the design of medical treatments based on “average patients” and “one-size fits all”. The concept of personalized medicine is not new, it is well documented that patients who present similar symptoms may have different underlying illnesses and may respond differently to medical intervention. However, the causes for these variant responses have not always been clear. As screening technologies continue to develop, it is becoming increasingly possible to use an individual’s genetic makeup or the molecular profile of the diseased tissue to guide the choice of medical treatment. All this is leading to a revolution in personalized medicine, which is driven by clinical efficacy and the re-imbursement pressures from payers who need to see appropriate health-economic impacts. Over the last 12 months, 28% of all new molecular entities and therapeutic biologics approved by the FDA were defined as personalized medicines whose label includes reference to biological markers that guides their administration [1].
Autologous cell therapies are arguably one of the most personalized forms of medicine, using a patient’s own cells to generate a bespoke product that is only administered back to the original donor. There are also good examples of autologous products in late stage clinical trials where patient stratification is used to increase therapeutic efficacy. However, as more allogeneic therapies are developed and the global cell therapy product pipeline increases, it is timely to look at how personalized medicine could further influence manufacture and disease management strategies.
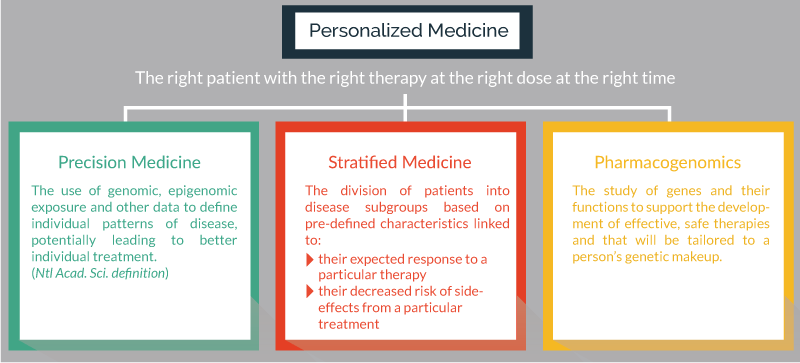
Figure 1. The concept of personalized medicine and the role of precision medicine, stratified medicine and pharmacogenomics
The role of personalized medicine for allogeneic therapies
The manufacture and supply of allogeneic therapies tends to follow similar production strategies, where a large batch of product is manufactured, formulated, filled, cryogenically stored and tested prior to shipment and administration. Batches of allogeneic products therefore tend to be of a predefined dose and administered identically to all patients using a pre-established dosing regimen. As a result, there are a number of opportunities to apply personalized medicine strategies to support the decision making about the timing and application of allogeneic cell and gene therapy products Figure 2. These include the development of screening tools to identify patient populations who will derive the most benefit from a cell therapy in preference to other treatment options Figure 2-1,the use of dosing strategies based on disease progression and/or likely responsiveness to a cell therapy Figure 2-2, the development of monitoring strategies for patients post-administration Figure 2-3, to allow physicians to progress with subsequent dosing based on the original treatment plan or to modify the plan due to a patient’s response to their current treatment.
There are of course challenges in applying this type of approach. Based on the final volume of the therapy there may be a need to produce different inventories of the product in order to allow the administration of different doses based on disease or patient characteristics. This investment decision is likely to require significant upfront costs but could be justified if the tailored administration improves the overall clinical effectiveness or has a positive effect on the reimbursement price potential. In the long term this would support the increased manufacture cost threshold associated with implementing a multi-product inventory strategy. The benefits of this approach can be evaluated in advance through careful health economic assessment. The success of personalized medicine also depends on matching the correct treatment to the individual needs of the patient. Therefore, developers would need to identify suitable biomarkers and companion diagnostic tests in order to establish the best therapeutic strategy for an individual patient. Ideally this would need to start early in the clinical process, even in the pre-clinical phase, in order to be able to relate biomarker expression to clinical outcome. This could show that the therapy is not only safe and effective, but also how it is best delivered and which patients will derive the most benefit. For the development of therapeutic biologics this approach has become commonplace, indeed, over 50% of all clinical trials involving biologics collect patient samples in order to aid biomarker development [2]. If simple biomarkers are identified, then this can make patient screening to decide on a therapeutic strategy relatively straight forward using simple immunoassays or genetic tests to identify likely responders. An example of this approach used for biologics is the screening of patients with metastatic colon cancer to identify individuals with a mutated form of the KRAS gene in their tumour cells. These patients don’t respond to treatments targeting anti-epidermal growth factor receptor such as cetuximab as the mutated KRAS protein continues to signal the cancer cells to divide despite the receptor inhibition [3].
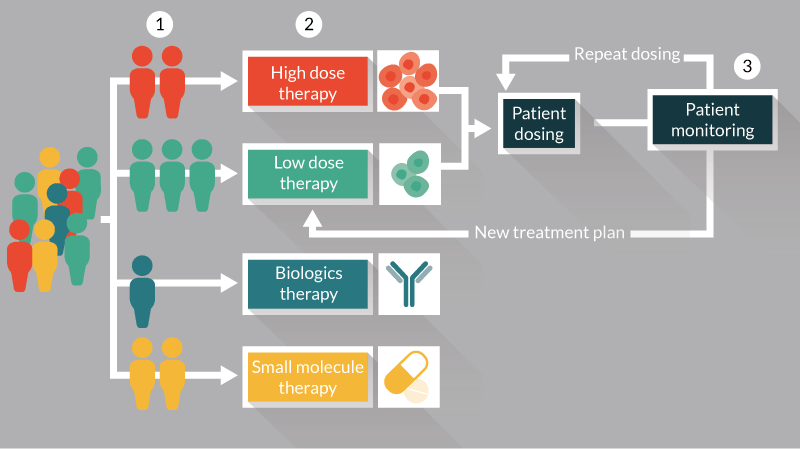
Figure 2. Opportunities for the applications of personalized medicine for allogeneic cell therapy manufacture and administration
Personalized medicine strategies could also be applied to decide on the timings of cell therapy administration including opportunities for pre-emptive interventions. For example, hematopoietic stem cell transplants are widely used to treat malignant and non-malignant haematological disorders. The treatment works by engrafting and reconstituting the host immune system. However, a severe and quite common life-threatening complication is the development of graft-versus-host disease (GVHD) where the allo-reactive donor T cells from the transplant begin to attack host tissues [4]. A number of allogeneic cell therapies using mesenchymal stromal/stem cells (MSC) are currently being developed to treat the acute form of GVHD (aGVHD) including remestemcel-L, which is approved for the treatment of pediatric aGVHD in several countries. The therapeutic mechanism of action of these MSC therapies is largely thought to be through immune modulatory effects, suppressing the allo-reactive T cells and promoting tissue repair through paracrine effects [5]. One of the difficulties in treating aGVHD is that the diagnosis usually relies of the presence of clinical symptoms. Therefore, it would be highly beneficial to be able to identify patient who are at high risk of aGVHD and design new treatment strategies, including early interventions, to prevent or control its onset. Unfortunately, to date there are no validated tests for patient stratification based on risk of developing aGVHD, but there are a large number of candidate biomarkers being investigated [6]. A number of these biomarkers could have the sensitivity and specificity to allow patient stratification based on likely unresponsiveness to first-line interventions such as systemic steroid treatment. In future, validation of these biomarkers could allow patient stratification to select those who would benefit most from alternative interventions such as MSC therapies to reduce mortality rates and prevent the development of refractory disease.
The role of personalized medicine for autologous therapies
For autologous therapies focused on clinically challenging targets such as solid tumors, personalized medicine could be used to identify biological correlates of predicted patient responses based on the tumor microenvironment. Examples of these therapies include purified and expanded tumor infiltrating lymphocytes (TIL) or the targeting of TIL’s to specific cancers using gene modified T-Cell receptor (TCR) or chimeric antigen receptors (CAR). This is an area of much interest and a number of CAR and TCR products targeting solid tumor antigens are currently under clinical investigation [7]. One of the challenges to targeting cell therapies at solid tumors is that the composition and characteristics of the microenvironment is highly variable and contributes significantly to treatment efficacy [8]. This heterogeneity has been demonstrated at the protein, gene, and epigenetic level and can lead to a reduction in the proliferative potential of the transplanted cells and their differentiation to an effector like phenotype [9]. The proliferation of TILs can also be impacted by the genomic characteristics of the cells, with reduced clinical efficacy in products containing cells with short telomeres [10]. Therefore, a holistic understanding of the genomic characteristics of the product, the inhibitory environment of the tumor and the crosstalk between the parenchyma and stroma is required when looking to identify factors which could be used to predict clinical efficacy. Commercially, autologous products also have higher associated cost of manufacture as they are low volume, bespoke and in the case of gene modified immunotherapies use GMP grade virus which is a significant component of their overall cost of goods [11]. Therefore, it would be advantageous to be able to screen patients at a more in depth level than currently used and implement stratification prior to embarking on a physically and commercially demanding treatment process.
The ability to stratify patients based on predicted response can be one of the biggest challenges to implementing personalized medicine strategies. A number of organizations are developing autologous CAR T-Cells therapies for the treatment of chronic lymphocytic leukaemia (CLL) using CD19 as a target [12-14]. These therapies have shown promise in the treatment of patients with relapsed and refractory forms of the disease, but complete remission is so far only observed in around 30% of patients treated [15]. The ability to stratify this patient population would increase overall responsiveness, but so far no patient or disease characteristics have been identified which can predict clinical outcome. However, as clinical data increases, it may be possible to use the current CLL cytogenetic screens to select patients for immunotherapy treatment. For example, there are a number complex karyotypic abnormalities that are linked to inferior progression free survival of CLL patients treated with biological therapeutics targeting B-cell malignancies [16]. These include monoallelic or biallelic deletion of the short arm of chromosome 17 (Del17p) which is found in 5-10% of patents and is the strongest predictor of rapid disease progression and low response rates to front-line therapy [17-18]. Interestingly, results from clinical trials using CD19 CAR T -cells have shown that patients with Del17p mutations can enter complete remission without experiencing any acute side effects [19]. Other prognostic markers for CLL are also being investigated. These include epigenetic marker such as aberrant hypermethylation of signaling pathway genes present in CLL [20], differential expression of micro-RNA markers which predict progression of CLL [21] and gene markers such as SKI and SLAMF1 which could be used to predict overall survival rates for CLL patients [22]. In the future, a better understanding of the role that epigenetics, differential gene expression profiles and cytogenetics play in the pathogenesis of CLL could allow more target disease management with preferential use of immunotherapies where efficacy benefits can be predicted.
Similar stratification strategies could also be used to reduce toxicity associated with some immunotherapies such as cytokine release syndrome (CRS). This life threatening complication occurs when cytokines associated with T cell engagement and proliferation such as IL- 6, IL-10 and interferon-γ are systemically released [23]. A certain level of cytokine release is expected following immunotherapy administration as a consequence of T cell activation and is linked to product efficacy. However, it is important to have strategies in place to monitor and manage CRS before severe complications emerge. If patients do suffer CRS, there are a number of potential treatment options including IL-6 blockade using humanized monoclonal antibody drugs such as tocilizumab which can result in the reversal of severe CRS symptoms [23]. However, treatment options have to be considered against the risk of inhibiting the activity of the T cell therapy which would impair the efficacy of the selected treatment plan. This would ideally be done in combination with post-administration residual disease monitoring. Together, these could be used as a quantitative prognostic tool to aid clinical decisions about the timing of any follow on treatment based on risk of disease relapse versus the potential for adverse patient response.
Personalized medicine and health economics
The concept of personalized medicine is to enhance patient care by ensuring they receive the most beneficial medicinal intervention during the optimum therapeutic window. As a consequence, personalized medicines should also lower healthcare costs by ensuring efficient use of drugs while maximising the benefit to the patient. This approach is particularly important for economically significant disease areas where the number of available drugs continually increases. For example, there were 26 new anticancer drugs launched between 2013 and 2015 into an expanding oncology field [24] with annual global sales of £55bn ($79bn) [25]. Similar early trends are being seen in the cell and gene therapy field with approximately 35 CAR-T cell therapy products in various stages of development for the treatment of B cell malignancies using CD19 as their target [26]. It is expected that within the next decade at least 8 of these will be commercially available, meaning there will be a requirement for mechanisms to guide clinicians in administering the most appropriate competing therapy to defined patient groups.
A 2015 report form the National Bureau of Economic Research which examined prices for anticancer drugs showed that the average price for a round of treatment is £45,000 ($66,000) for which patients can expect an average survival benefit of 0.46 years [27]. For cancers such as leukaemia, these average prices can be even higher ranging from £32,000 per year for Omacetaximine to £97,000 for treatment with Ponatinib [28] (Figure 3). It is highly likely that cell and gene therapy products will be even more expensive provided they can demonstrate increase patient benefit or even curative potential, as this will be required to offset their expensive cost of manufacture. A recent study by the UK National Institute for Health and Care Excellence [29] (NICE) looked at the fitness-for-purpose of their decision framework for assessing the reimbursement potential for cell and gene therapies. The study looked at the health economics for a hypothetical CD19 CAR T-cell therapy used to treat acute lymphoblastic leukemia (ALL). Two scenarios where modelled where the therapy was used either as a bridging product to induce short term remission prior to a hematopoietic stem cell transplant or with curative intent. The study demonstrated that in the absence of uncertainty in the supporting evidence, prices as high as £350,000 and £530,000 were feasible for the bridge and curative intent scenarios respectively. However, realistically at the time of launch the uncertainty would be too high to secure such prices and managed entry agreements would be required to absorb uncertainty and mitigate risks for the payer [29]. Therefore, personalizing these high cost therapies to ensure they are administered to patient who will derive the most benefit will reduce the uncertainty and boost the price potential.
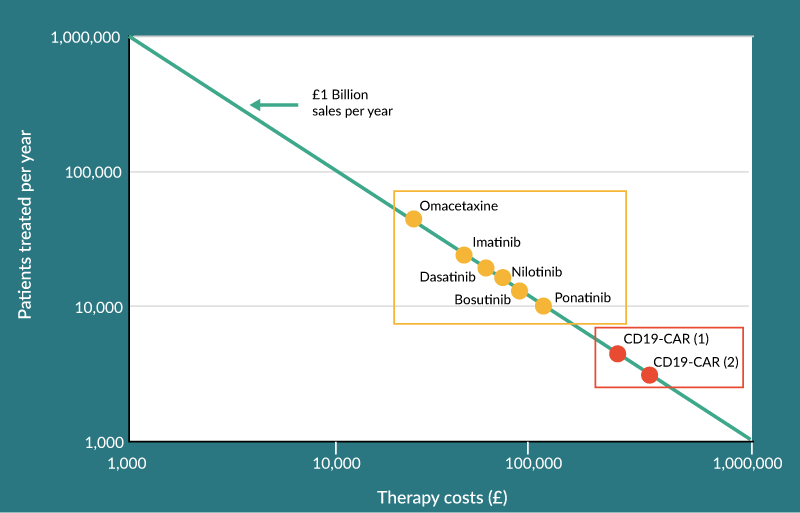
Figure 3. Using personalized medicine to tailor cell therapy administration will increase price potential allowing blockbuster returns while maximizing patient benefit. Current leukemia drugs which offer short term treatment are show in orange compared to two hypothetical scenarios showing potential optimum reimbursement levels for the CD19-CAR T-cell therapy used as a bridging product (CD19-CAR [1]) and as a curative product (CD19-CAR [2]).
Future perspective – Personalized medicine and gene editing
Traditional gene modified therapies use viral vectors to stably transfer exogenous DNA into the genome. This approach has proven very successful for the large range of products currently undergoing clinical trials, but can be limited by a lack of control over the site(s) of genomic integration, the potential for insertional mutagenesis and unforeseen effects on genes surrounding the insertion site [30]. These unwanted characteristics could be overcome by emergent gene editing technologies such as zinc finger nucleases, transcription activator-like effector nucleases (TALENs), clustered regulatory interspaced short palindromic repeat nucleases (CRISPR) and meganucleases. These technologies have the ability to induce double stranded DNA breaks at precise locations in the genome to allow the targeted insertion of therapeutic transgenes. The main advantage of this targeted approach is that donor genes can be inserted directly into their endogenous locus allowing them to be controlled by their natural promotor elements [31]. The use of gene editing technologies is increasing rapidly and they are now being used in preclinical studies to treat a range of diseases including Duchenne muscular dystrophy [32], epidermolysis bullosa [33], X-linked severe combined immunodeficiency (X-SCID) [34] and cystic fibrosis [35]. While it is still early days for these technologies their potential to be used as personalized medicines is already being explored. For example, cystic fibrosis which can be caused by almost 2000 mutations in the gene encoding the Cystic Fibrosis Transmembrane conductance Regulator (CFTR) protein [36]. These mutations can have different biological consequences ranging from impaired mucociliary clearance [37] through to dysregulation of toll-like receptors which affects the innate immunity [38]. The ability to use personalized medicine approaches to select patients for gene editing therapies to correct specific mutations and restore biological function is therefore very attractive. Indeed, it has already been shown that the p.F508del mutation which affects around 70% of cystic fibrosis patients can be corrected in lung epithelial cells using the TALENs system [39], although in vivo delivery of cells following gene editing may present an ongoing and significant challenge.
Gene editing technologies also offer the exciting potential to treat diseases by knocking out endogenous genes or permanently disrupting their function. This is an area of increased activity and several phase 1 clinical trials have been conducted to treat disease such as HIV using zinc finger nucleases for site specific modification of CD4+ T cells [40]. The majority of these studies have looked to knockout the gene for the CCR5 co-receptor to reduce the ability of HIV to infect the cells. These studies have demonstrated safe engraftment and persistence of the cells with a mean half-life of up to 48 weeks and an overall reduction in detectable HIV RNA in some patients [40]. Other studies have looked at using gene editing to treat HIV infected cells by removing the viral genome, targeting the long terminal repeats that flank the integrated viral sequence [41]. Again it is early days for these therapies but as the technology improves there could be several new treatment options using gene editing to treat diseases such as HIV with patient treatment optimized using personalized medicine approaches.
Conclusion
The cell and gene therapy field is going through a stage of enormous growth with increased global investment, more products entering pivotal clinical trials and an increase in the number of diseases being targeted. Looking ahead, the ability to tailor the administration of cell and gene therapies to maximise patient and reimbursement impact will further aid this growth. In the short term, this will involve companies bearing the costs associated with the development of diagnostics to aid stratification and potential increased manufacturing costs for multiple product inventories. However, the ability to ensure that often costly manufacturing processes are targeted at patients who will derive the most benefit will have positive commercial implications in the mid to long term. The significant upside is having a portfolio of more efficacious products with more commercially favorable clinical and reimbursement potential. It is therefore inevitable that the ability to use advanced therapies for customized healthcare will lead to the development of new business and medical treatment models for the cell and gene therapy field with personalized medicine applications at their core.
References
1. Personalized Medicine Coalition. 2015 progress report – Personalised Medicine at FDA (2015).
2. Personalized Medicine Coalition. Personalized Medicine – By the Numbers (2015).
3. Lièvre A, Bachet JB, Le Corre D et al. KRAS mutation status is predictive of response to cetuximab therapy in colorectal cancer. Cancer Res. 2006; 66(8), 3992-5. CrossRef
4. Blazar BR, Murphy WJ, Abedi M. Advances in graft-versus-host disease biology and therapy. Nat Rev Immunol. 2012; 12(6), 443-58. CrossRef
5. Caimi PF, Reese J, Lee Z, Lazarus HM. Emerging therapeutic approaches for multipotent mesenchymal stromal cells. Curr Opin Hematol. 2010; 17(6),505–13. CrossRef
6. Paczesny S, Raiker N, Brooks S, Mumaw C. Graft-versus-host disease biomarkers: omics and personalized medicine. Int J Hematol. 2013; 98(3), 275-92. CrossRef
7. Christian S. Hinrichs CS and Rosenberg SA. Exploiting the curative potential of adoptive T-cell therapy for cancer. Immunol Rev. 2014; 257(1), 56–71. CrossRef
8. Kakarla S & Gottschalk S. CAR T cells for solid tumors: armed and ready to go? Cancer J. 2014; 20(2), 151–5.
9. Burrell RA, McGranahan N, Bartek J, Swanton C. The causes and consequences of genetic heterogeneity in cancer evolution. Nature 2013; 501, 338–45. CrossRef
10. Zhou J, Shen X, Huang J et al. Telomere length of transferred lymphocytes correlates with in vivo persistence and tumor regression in melanoma patients receiving cell transfer therapy J Immunol. 2005; 175(10), 7046-52. CrossRef
11. Callens S, Hassan J, McCoy R and Ward S. Cell and gene therapy manufacturing: the necessity for a cost-based development approach. Cell Gene Therapy Insights 2016; 2(1), 115-120. CrossRef
12. Brentjens R, Yeh R, Bernal Y, Riviere I, Sadelain M. Treatment of chronic lymphocytic leukemia with genetically targeted autologous T cells: case report of an unforeseen adverse event in a phase I clinical trial. Mol Ther 2010; 18(4), 666-668. CrossRef
13. Porter DL, Kalos M, Zheng Z, Levine B, June C. Chimeric antigen receptor therapy for B-cell malignancies. J Cancer 2011; 2, 331-332. CrossRef
14. Kochenderfer JN, Wilson WH, Janik JE, et al. Eradication of B-lineage cells and regression of lymphoma in a patient treated with autologous T cells genetically engineered to recognize CD19. Blood 2010; 116(20), 4099-4102. CrossRef
15. Porter DL, Hwang WT, Frey NV et al. Chimeric antigen receptor T cells persist and induce sustained remissions in relapsed refractory chronic lymphocytic leukemia. Sci Transl Med 2015; 7(303), 303ra109. CrossRef
16. Thompson PA, O’Brien S, Wierda WG et al. Complex karyotype is a stronger predictor than del(17p) for an inferior outcome in relapsed or refractory chronic lymphocytic leukemia patients treated with ibrutinib-based regimens. Cancer. 2015; 121(20), 3612-21. CrossRef
17. Badoux XC, Keating MJ, Wierda WG. What is the best frontline therapy for patients with CLL and 17p deletion? Curr Hematol Malig Rep. 2011; 6(1), 36-46. CrossRef
18. Heerema N, Muthusamy N, Ruppert A et al. Prognostic Significance Of Cytogenetic Complexity and Del(17p) At Diagnosis Of Chronic Lymphocytic Leukemia (CLL) Blood 2013; 122(21), 1321.
19. Porter DL, Levine BL, Kalos M et al. Chimeric antigen receptor modified T cells in chronic lymphoid leukemia. N Engl J Med. 2011; 365, 725-33. CrossRef
20. Pei L, Choi JH, Liu J et al. Genome-wide DNA methylation analysis reveals novel epigenetic changes in chronic lymphocytic leukemia. Epigenetics. 2012; 7(6), 567-78. CrossRef
21. Calin GA, Ferracin M, Cimmino A et al. A MicroRNA signature associated with prognosis and progression in chronic lymphocytic leukemia. N Engl J Med. 2005; 353, 1793–1801. CrossRef
22. Schweighofer CD, Coombes KR, Barron LL et al. A two-gene signature, SKI and SLAMF1, predicts time-to-treatment in previously untreated patients with chronic lymphocytic leukemia. PLoS One. 2011; 6:e28277. CrossRef
23. Maude SL, Barrett D, Teachey DT, Grupp SA. Managing cytokine release syndrome associated with novel T cell-engaging therapies. Cancer J. 2014; 20(2), 119-22. CrossRef
24. Price and Value of Cancer Drugs. Memorial Sloan Kettering Cancer Centre report (2015). (www.mskcc.org)
25. Top 20 therapeutic classes by global pharmaceutical sales in 2015 (in billion U.S. dollars). Statista report (2015). (www.statista.com)
26. CD19 Therapeutics Market, 2016 – 2030. ReportLinker analysis (2016) (www.reportlinker.com)
27. Pricing in the marker for anticancer drugs. National Bureau of Economic Research Report (2015) (http://www.nber.org/papers/w20867.pdf)
28. Abboud C, Berman E, Cohen A et al. The price of drugs for chronic myeloid leukemia (CML) is a reflection of the unsustainable prices of cancer drugs: from the perspective of a large group of CML experts. Blood. 2013; 121(22), 4429-4442. CrossRef
29. Crabb N and Steven A. Exploring the assessment and appraisal of regenerative medicines and cell therapy products. National Institute for Health and Care Excellence report (2016).
30. Maeder M and Gersbach C. Genome-editing Technologies for Gene and Cell Therapy. Mol Ther. 24(3), 430-446. CrossRef
31. Li H, Haurigot V, Doyon Y et al. In vivo genome editing restores haemostasis in a mouse model of haemophilia. Nature. 2011; 475(7355), 217–221. CrossRef
32. Ousterout DG, Perez-Pinera P, Thakore PI et al. Reading frame correction by targeted genome editing restores dystrophin expression in cells from Duchenne muscular dystrophy patients. Mol Ther; 2013; 21(11), 1718–1726. CrossRef
33. Osborn MJ, Starker CG, McElroy AN et al. TALEN-based gene correction for epidermolysis bullosa. Mol Ther. 2013; 21(6): 1151–1159. CrossRef
34. Genovese P, Schiroli G, Escobar G et al. Targeted genome editing in human repopulating haematopoietic stem cells. Nature. 2014; 510(7504): 235–240. CrossRef
35. Crane AM, Kramer P, Bui JH et al. Targeted correction and restored function of the CFTR gene in cystic fibrosis induced pluripotent stem cells. Stem Cell Reports. 2015; 4(4): 569–577. CrossRef
36. Corvol H, Thompson KE, Tabary O, le Rouzic P, Guillot L. Translating the genetics of cystic fibrosis to personalized medicine. Transl Res. 2016; 168, 40-49. CrossRef
37. Hoegger MJ, Fischer AJ, McMenimen JD et al. Cystic fibrosis. Impaired mucus detachment disrupts mucociliary transport in a piglet model of cystic fibrosis. Science. 2014; 345(6198): 818-822. CrossRef
38. Sallenave JM. Phagocytic and signaling innate immune receptors: are they dysregulated in cystic fibrosis in the fight against Pseudomonas aeruginosa. Int J Biochem Cell Biol. 2014; 52: 103-107. CrossRef
39. Wong AP, Bear CE, Chin S et al. Directed differentiation of human pluripotent stem cells into mature airway epithelia expressing functional CFTR protein. Nat Biotechnol. 2012; 30(9): 876-882. CrossRef
40. Tebas P, Stein D, Tang WW et al. Gene editing of CCR5 in autologous CD4 T cells of persons infected with HIV. N Engl J Med. 2014; 370(10): 901-910. CrossRef
41. Hu W, Kaminski R, Yang F et al. RNA-directed gene editing specifically eradicates latent and prevents new HIV-1 infection. Proc Natl Acad Sci.. 2014; 111(31): 11461–11466. CrossRef
This work is licensed under a Creative Commons Attribution- NonCommercial – NoDerivatives 4.0 International License.
Finanancial and Competing Interests Disclosure
Cell & Gene Therapy Catapult is an Innovate UK programme.
The authors are employees of Cell and Gene Therapy Catapult.
No writing assistance was utilized in the production of this manuscript.
AFFILIATIONS
Damian Marshall
Head of Analytical Development at Cell Therapy Catapult
Michaela Sharpe
Head of Non-Clinical Safety and Immunotherapy Strategy, Cell & Gene Therapy Catapult
Dr Stephen J Ward
Chief Operating Officer, Cell & Gene Therapy Catapult