Advances in automation for the production of clinical-grade mesenchymal stromal cells: the AUTOSTEM robotic platform
Cell Gene Therapy Insights 2017; 3(8), 739-748.
10.18609/cgti.2017.073
Stem cell-based therapies are a central element of regenerative medicine and provide new treatment modalities for chronic and life-threatening conditions. Mesenchymal stem/stromal cells (MSCs) represent an important technology in regenerative medicine, although less developed with respect to clinical translation than hematopoietic stem cells (HSCs). MSC therapies may be based on the potential of the cells to differentiate to mesenchymal lineages or on their paracrine effects on host tissue. Both autologous and allogeneic applications are possible, the latter enabled by the low immunogenicity of the cells. Although stem cell therapy holds much promise for the treatment of chronic and debilitating diseases, there are still many obstacles to be overcome. In addition to the compelling need to generate strong and unambiguous clinical evidence, there are major technical gaps that must be filled. Chief among these is the development of manufacturing platforms for cell products that are efficient, cost effective and reproducible. Automated, robotic and closed production systems will provide the most efficient manufacturing strategy. Here we describe advances in automation for the clinical-scale production of MSCs and challenges associated with translating from lab-scale to automated large-scale manufacturing processes.
MESENCHYMAL STROMAL CELLS: CLINICAL POTENTIAL
The prevalence of chronic diseases worldwide and the transition towards an older population lead to a greater demand for hospital care and complex medical interventions. The economic and societal burdens associated with this trend underscore the need for new disruptive technologies such as regenerative medicine. A recent analysis undertaken by the Healthcare Cost and Utilization Project indicates that hip and knee replacements for the treatment of osteoarthritis (OA) are the most common surgical procedures carried out in the USA [1] . Data from the 2013 Global Burden of Disease (GBD) survey also reported an estimated 242 million people worldwide suffering from OA of the hip and/or knee with an accumulated 13 million years lived with disability [2] . Furthermore, GBD mortality figures for 2015 also indicated that 31% of deaths were associated with cardiovascular disease further highlighting the need to move beyond current care [3] . Stem cell-based therapies are a central element of regenerative medicine and provide new treatment modalities for chronic and life-threatening conditions. The impact of this technology is evidenced by the fact that over a million procedures for autologous or allogeneic hematopoietic stem cell (HSC) transplantation for the treatment of malignant and non-malignant conditions have been performed [4,5] .
Mesenchymal stem/stromal cells (MSCs) represent an important technology in regenerative medicine, although less developed with respect to clinical translation than HSCs. MSCs were originally isolated from bone marrow as rapidly adherent, fibroblastic-like cells that were derived from a single cell colony-forming unit with the capacity to form bone, cartilage and adipose tissue in vivo [6] and differentiating to these lineages in vitro [7] . In 2006, the International Society for Cellular Therapy (ISCT) proposed criteria to describe human MSCs suggesting that: 1) the cells should be plastic-adherent in vitro; 2) show surface expression of CD105, CD73 and CD90 but not express markers associated with hematopoietic cells; and 3) differentiate to osteoblasts, adipocytes and chondrocytes [8]. MSC therapies may be based on the potential of the cells to differentiate to mesenchymal lineages or on their paracrine effects on host tissue [7,9] . Both autologous and allogeneic applications are possible, the latter enabled by the low immunogencity of the cells [10] . One of the first reports on the clinical use of MSCs described a tissue engineering approach for reconstruction of large bone segments in three patients using autologous bone marrow-derived MSCs loaded on a hydroxyapatite scaffold [10] . In a follow-up assessment 6–7 years later, integration of the implant was maintained and no fractures were observed in the implant zone [12] . Positive effects of direct, scaffold-free delivery of MSCs for modulation of osteoarthritis development in a caprine model was first reported in 2003 [13] .
Clinical assessment of MSCs derived from a number of sources, including bone marrow, adipose and umbilical cord tissue has developed significantly since that time. By 2010, over 5,000 patients in 101 clinical trials were treated with MSCs and approximately 85% of these involved ex vivo culture-expanded cells [14] . A broad spectrum of conditions was assessed, from immune-mediated to neurological diseases, although musculoskeletal indications represented the largest group. By 2016, 493 MSC clinical trials were registered but few of these have reached an advanced stage of assessment with just 26 Phase 3 and three Phase 4 trials reported [15,16] . However, translation to clinical practice has also been slow. Osiris Therapeutics first received approval for an MSC-based therapy in 2012: Prochymal for the treatment of childhood acute graft-vs-host disease (aGvHD) in Canada and New Zealand. More recently, Mesoblast received market approval in Japan for TEMCELL for the treatment of aGvHD in children and adults. In 2017, Mesoblast also reported promising Phase 3 interim results for their allogeneic MPC-150-IM product for chronic heart failure [17] . Positive results of a Phase 3 study using an expanded allogeneic adipose-derived stem cell product from Tigenix (Cx601) in Crohn’s disease were also described recently [18] . Cx601 was shown to be effective and safe in the treatment of complex perianal fistulas in patients with Crohn’s who had not responded to conventional treatments.
CHALLENGES IN TRANSLATING MSC PRODUCTION
From lab-scale to automated large-scale manufacturing processes
As market approvals progress over the next few years, manufacturing competence with efficiencies of scale, reproducibility and cost will become a critical bottleneck. To facilitate the transition to broad clinical application, significant manufacturing innovation is needed which will take account of the inherent complexity of cell-based therapeutics. To date, production of cell therapeutics has relied on manual protocols, giving rise to concerns of contamination, batch to batch variability and high labor costs. To address these challenges, a focus on highly automated, robotic manufacturing platforms is required. The use of closed automated systems decreases the likelihood of failures due to human error and leads to standardization of the production process and culture conditions to generate a consistently reproducible cell product that meets regulatory standards for release and scale for clinical use. Regulatory compliance is also a driver for increasing automation because of comprehensive centralized recording and storage of process data. In 2014, Trainor et al. commented that a “transformation needs to take place in the way we manufacture and deliver” autologous adult stem cell therapies [19] . These authors proposed that de-centralized automated production at the point of care would address this issue and interestingly proposed that this could be facilitated by a “standard business franchising model”. It seems clear that the promise of either allogeneic or autologous MSC therapy will remain unmet until advanced methods for automated, scalable, closed and large-scale expansion methods become available. The AUTOSTEM project aims to develop and validate a closed, aseptic, ‘donor-to-patient’ automated, modular system for the Good Manufacturing Practice (GMP) production of therapeutic MSCs [20,21] .
While discussion about automation has been elevated throughout the cell therapy industry, there is currently no unified understanding of the term and it is often used to describe different approaches. For example, it can refer to stand-alone devices such as pipetting robots or individual laboratory equipment that automates a specific process step. The cultivation of cells in bioreactors allows control of parameters in a semi-automated manner. There are many bioreactor technologies and platforms available on which successful MSC production has been reported [22–24] . While some of the solutions for semi-automation include specialized equipment for expansion in a closed system [25] , another approach is to mimic manual processes such as media exchange in T-Flasks by robot-assisted automation [26] . However, solutions that provide a full automation of the entire process chain are still rare. Industries manufacturing cells for autologous therapy would benefit significantly from this approach as the production is underpinned by high risks and regulatory restrictions. It is also clear that automated production of MSCs for allogeneic therapies would expedite the translational process.
Although it is appreciated that automation has the potential to support the cell therapy sector significantly, the transition to full automation still faces many challenges. For example, even though individual automated laboratory devices are commercially available, there is a lack in interface standards for interconnection of the devices. At the same time the consistency of data transfer is key for more complex automation strategies that rely on manifold devices and sensors. Another issue that arises for processes with increasing complexity is the technical robustness of all individual devices, and the overall process chain as the risk for failure is multiplied by every additional system added. For this reason, systems with decreased complexity and rigid processes are more prevalent. However, these systems only partially address automation and the field of cell therapy is still rather young and therefore changing rapidly. It is also clear that technical systems need to allow a certain degree for flexibility, otherwise manufacturers face the risk that their equipment will rapidly become outdated. In addition to technical challenges for automation of cell therapy production, there is some apprehension about the use of electronic computerized systems and regulatory requirements relating to data integrity, consistency, storage and protection. In this regard the requirements set out in Annex 11 of the EudraLex Volume 4 are of high importance [27] .
There are indeed many advantages for fully automated systems that provide a compelling case for such a transition from the primarily manual processes currently in use in the field. These include:
- Decreased risks for contamination by transition of high-risk processes such as tissue manipulation or open cell handling to closed, automated processes.
- Avoidance of human errors, for example mix-up of materials.
- High level of process control beyond the capabilities of a human operator.
- Generation of more data to facilitate a better process understanding.
- Higher throughput.
- Simplification of Track-and-Trace for every cell batch in the form of finely-tuned documentation.
In order to meet these challenges, the AUTOSTEM project aims to develop a closed robotic platform to achieve fully automated manufacture of MSCs that requires no direct human interaction with the product [20,21] . The platform builds on technology developed for the ‘StemCellFactory’ for fully automated generation and expansion of standardized induced pluripotent stem cells which consisted of a robotic production suite above an area with capacity for both ambient and cooled storage for materials required for a production campaign [28,29] . The AUTOSTEM cell factory will consist of three individual, interlinked areas to facilitate clinical production of MSCs according to GMP guidelines (Figure 1) placed on top of the customized storage area. The transition area will enable wipe-down and loading of the platform by operators with bar-coded materials between manufacturing campaigns prior to gas sterilization. All closed processes including receipt of the source tissue, MSC seeding and expansion in selected bioreactors, sampling and in-process analyses, and cryopreservation will be performed in a Grade D environment. The modular design in this area will enable incorporation of selected bioreactor formats.
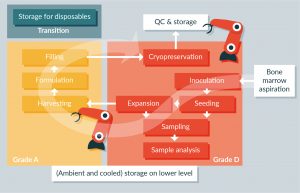
Figure 1: Overview of the automated manufacturing process performed by the AUTOSTEM cell factory for production of adherent MSCs. Cell processing in the platform is performed in two individual chambers with a grade D and a grade A background. This partition enables processing of cells in a closed and open manner as required. Both chambers are equipped with a robot arm that assists the process by handling of disposables and implementation of all manipulation steps. Bone marrow aspirate is harvested with a novel device and transferred into the AUTOSTEM pipeline in a completely closed manner. The bone marrow is used for inoculation of a two-stage bioreactor process in which MSCs are isolated from the bone marrow or other source tissues initially and then expanded further. Quality control is applied by sampling regularly from the bioreactors. After completion of the expansion phase, the cell suspension is transferred automatically into a grade A environment where it can be handled openly. After harvesting, cells are formulated and transferred back to the Grade D environment for cryopreservation followed by manual transfer from the platform.
Higher throughput will be enabled by minimization of contamination events, continuous 24/7 capacity and the possibility of using multiple bioreactors of any format to stagger production of batches from working cell banks. The design offers the possibility that multiple autologous patient batches could be run simultaneously but would depend on validation that cross-contamination does not occur. This may be enabled in future iterations of the platform.
Source tissue, for example bone marrow harvested from patients or donors, will be delivered into the pipeline in a sterile, closed manner through custom-designed tube-sets and the environment in the pipeline bioreactors will be closely monitored to ensure optimal conditions are maintained throughout. Open processes such as cell harvest and formulation prior to cryopreservation will be performed in a Grade A environment. The output of the pipeline will be clinical doses of MSCs that will comply with accepted release criteria.
In the case of the AUTOSTEM process, the risk of contamination is reduced for ‘closed’ steps of the culture process including transfer and processing of the source tissue, cell seeding, sampling and analytical assays. Closed transfer of the MSC product to the Grade A environment is also enabled for automated harvesting, formulation and filling for cryopreservation or immediate delivery to patients without human intervention. Tissue procurement is recognised as presenting a major risk of contamination in cell therapy protocols [30] . However, the development of strict skin decontamination regimens prior to tissue harvesting and novel, closed harvesting devices to enable transfer of the source tissue begin to address this issue [31,32] .
The AUTOSTEM platform follows a fully closed, fully integrative approach. In order to achieve a high technical robustness, industry standard programmable logic controllers are used. A specially developed and modular software that moderates the communication between the devices and a centralized control level will ensure consistency of data collection and storage. The platform offers the possibility of generating comprehensive records on cell product history: since all manipulation steps are tracked with a time stamp, a finely meshed documentation system can be generated for each individual production campaign. Cell growth, environmental conditions, operation times and the duration of individual processing steps are all tracked. For example, if a cell vial containing a formulated product is picked up by the robot and transferred to a different position, the event is recorded together with a time stamp. The system will therefore enable comprehensive retracing of all process steps.
It is sometimes suggested that process flexibility is a disadvantage in the manufacture of therapeutic products. While this may be the case for established processes in pharmaceutical manufacturing, the situation is slightly different for cell therapies. The cell therapy industry is still comparatively young and manufacturing processes may require optimization as experience grows and new technical improvements become available. We have seen these evolutions in production of biopharmaceuticals in recent years. The majority of cell therapies are still moving through clinical testing with adaptations in process and scale in development as progress is made towards Phase 4 testing. As the field progresses and these processes are further optimized, flexible, automated systems that can be easily adapted are required.
Our approach includes process development as well as the utilization of established technology such as bioreactors and carrier-based cell expansion. It is appreciated that flexibility needs to be incorporated into process development and the use of a robot arm for handling disposables at each step facilitates this. At the same time the approach addresses the need for increased throughput, achieved by evolution to a two-step process of multi-liter scale. As the platform is modular, different bioreactors of choice can be incorporated, thereby allowing modification of the format and scale when required.
Of course, as processes are transferred to automation, it is crucial to ensure that the product does not change. As there is not yet a full understanding of the interactions between the process and the product, all elements require close monitoring. This can be achieved by choosing a “Quality by Design” (QbD) approach, which is well appreciated in industrial cell culture processes such as the production of biopharmaceuticals [33] . An important part of the QbD approach is the understanding of how different parameters influence the process and therefore the product. The boundaries for these parameters have to be well defined and ensure that the process is operated within the process design space. This guarantees that production is safe and compliant with regulatory requirements. Within the cell therapy industry, the conviction that QbD will secure the quality of cell products is gaining traction [34]. This approach also helps to make the transition from manual processing to automation safe and knowledge-based. Therefore, the AUTOSTEM platform delivers the tools necessary for monitoring and tracking of process parameters and facilitates close control of the process. Additionally, it helps to build a data record which can be assessed using big data analysis. Cell culture conditions, processing steps and operation times are all tracked throughout the processing, thereby generating a comprehensive summary of the cell culture history. Finally, automation helps to control these parameters more closely and execute the process steps in a highly repeatable manner. For example, the duration of filling vials with the formulated cell suspension for cryopreservation will be far more reproducible compared to manual operations.
It is indeed necessary to have a complete understanding of process parameters. To this end, the development of defined and robust functional assays for both product and process analytical technologies (PAT) is required. This is a fundamental aspect of the QbD approach [35] . Many PAT tools, such as online monitoring of temperature, pH and partial oxygen pressure, are well established for bioprocessing. There are also sensors that give more complex readouts, such as those based on near infrared or Raman spectroscopy [36], but these technologies are not yet well established.
Additionally, extensive offline analytics for cell counting, assessment of metabolites, ions and osmolality are available. However, non-invasive high-quality PAT tools are very important to facilitate a better process understanding. For this reason the AUTOSTEM project also includes the development of a novel PAT sensor. A disposable semiconductor sensor probe will be mounted into the bioreactor for in-line process control [37,38] . The electrochemical-based sensor technology allows observation of multiple biological and chemical parameters simultaneously. In this way, the AUTOSTEM platform will support the generation of more online data beyond the recording of pH, pO2 and temperature.
TRANSLATIONAL INSIGHT
Although the fields of regenerative medicine in general, and stem cell therapy in particular, hold much promise for the treatment of chronic and debilitating diseases, there are still many obstacles to be overcome. In addition to the compelling need to generate strong and unambiguous clinical evidence, there are major technical gaps that must be filled. Chief among these is the development of manufacturing platforms for cell products that are efficient, cost effective and reproducible. Because of the complex nature of these biological products it seems more likely that dispersed manufacturing models will prevail. Production campaigns that rely on multiple human manipulations at any point will have an inherent risk of product loss due to costly contamination events.
Automated, robotic and closed production systems, with built-in flexibility in relation to individual processing steps and complete protection of the cell product from operator-derived contamination, will provide the most efficient manufacturing strategy. The AUTOSTEM stromal cell factory represents such a model. This will create the template for the GMP cell production facility of the future. The flexibility of the AUTOSTEM pipeline will not only achieve scale, but also wide applicability with its inbuilt modularity. As cell processing technology improves, the system can be adapted and reengineered, thus ensuring that it is always fit for purpose. It can be used for the manufacture of a broad spectrum of cell types, including autologous and allogeneic products. Autologous production could be implemented through a small-scale single bioreactor format and allogeneic use through multiple bioreactors for staggered production of batches from working cell banks. It will lead to reduced labor costs and reduced consumption of reagents, thus contributing to a reduction of cost of goods.
The development of the regenerative medicine industry depends critically on the design and implementation of manufacturing platforms such as the AUTOSTEM cell factory. It will take the field far beyond the research laboratory and much closer to marketability and delivery. It will move the cell therapy field forward to a point of realizable value for patients, clinicians and industry.
FINANCIAL & COMPETINGINTERESTS DISCLOSURE
The AUTOSTEM project was funded by the European Union’s Horizon 2020 research and innovation program under Grant Agreement No. 667932. Fraunhofer IPT was funded for the StemCellFactory project by the EU’s European Regional Development Fund and the German Federal State North Rhine-Westphalia (NRW) Ziel2.NRW and ExzellenzNRW research cluster. No writing assistance was utilized in the production of this manuscript.
REFERENCES
1. Kozma CM, Slaton T, Paris A, Edgell ET. Cost and utilization of healthcare services for hip and knee replacement. J. Med. Econ.2013; 16(7): 888–96.
CrossRef
2. Osteoarthritis: A Serious Disease 2016: Website
3. WHO Cardiovascular Diseases: Website
4. Gratwohl A, Pasquini MC, Aljurf M et al. One million haemopoietic stem-cell transplants: a retrospective observational study. Lancet Haematol. 2015; 2(3): e91–100.
CrossRef
5. Singh AK, McGuirk JP. Allogeneic Stem Cell Transplantation: A Historical and Scientific Overview. Cancer Res. 2016; 76(22): 6445–51.
CrossRef
6. Friedenstein AJ, Chailakhjan RK, Lalykina KS. The development of fibroblast colonies in monolayer cultures of guinea-pig bone marrow and spleen cells. Cell Tissue Kinet. 1970; 3: 393–403.
7. Barry F, Murphy M. Mesenchymal stem cells in joint disease and repair. Nat. Rev. Rheumatol. 2013; 9(10): 584–94.
CrossRef
8. Dominici M, Le Blanc K, Mueller I et al. Minimal criteria for defining multipotent mesenchymal stromal cells. The International Society for Cellular Therapy position statement. Cytotherapy 2006; 8(4): 315–7.
CrossRef
9. Vizoso FJ, Eiro N, Cid S, Schneider J, Perez-Fernandez R. Mesenchymal Stem Cell Secretome: Toward Cell-Free Therapeutic Strategies in Regenerative Medicine. Int. J. Mol. Sci. 2017; 18(9): E1852.
CrossRef
10. Lohan P, Coleman CM, Murphy JM, Griffin MD, Ritter T, Ryan AE. Changes in immunological profile of allogeneic mesenchymal stem cells after differentiation: should we be concerned? Stem Cell Res. Ther. 2014; 5(4): 99.
CrossRef
11. Quarto R, Mastrogiacomo M, Cancedda R et al. Repair of large bone defects with the use of autologous bone marrow stromal cells. N. Engl. J. Med. 2001; 344(5): 385–6.
CrossRef
12. Marcacci M, Kon E, Moukhachev V et al. Stem cells associated with macroporousbioceramics for long bone repair: 6- to 7-year outcome of a pilot clinical study. Tissue Eng. 2007; 13(5): 947–55.
CrossRef
13. Murphy JM, Fink DJ, Hunziker EB, Barry FP. Stem cell therapy in a caprine model of osteoarthritis. Arthritis Rheum. 2003; 48(12): 3464–74.
CrossRef
14. Ankrum J, Karp JM. Mesenchymal stem cell therapy: Two steps forward, one step back. Trends Mol. Med. 2010; 16(5): 203–9.
CrossRef
15. Trounson A, McDonald C. Stem Cell Therapies in Clinical Trials: Progress and Challenges. Cell Stem Cell. 2015; 17(1): 11–22.
CrossRef
16. Squillaro T, Peluso G, Galderisi U. Clinical Trials With Mesenchymal Stem Cells: An Update. Cell Transplant. 2016; 25(5): 829–48.
CrossRef
17. Mesoblast. MPC-150-IM Phase 2 trial 2017: Website
18. Panes J, Garcia-Olmo D, Van Assche G et al. Expanded allogeneic adipose-derived mesenchymal stem cells (Cx601) for complex perianal fistulas in Crohn’s disease: a phase 3 randomised, double-blind controlled trial. Lancet 2016; 388(10051): 1281–90.
CrossRef
19. Trainor N. PA, Smith T. Rethinking clinical delivery of adult stem cell therapies. Nature Biotechnol. 2014; 32: 729–35.
CrossRef
20. Rafiq QA, Twomey K, Kulik M et al. Developing an automated robotic factory for novel stem cell therapy production. Regen. Med. 2016; 11(4): 351.
CrossRef
21. AUTOSTEM: Website
22. Rafiq QA, Coopman K, Hewitt CJ. Scale-up of human mesenchymal stem cell culture: Current technologies and future challenges. Curr. Opin. Chem. Eng. 2013; 2(1): 8–16.
CrossRef
23. Placzek MR, Chung I-M, Macedo HM et al. Stem cell bioprocessing: fundamentals and principles. J. Royal Soc. Int. 2009; 6(32): 209–32.
CrossRef
24. Jossen V, Pörtner R, Kaiser SC, Kraume M, Eibl D, Eibl R. Mass Production of Mesenchymal Stem Cells – Impact of Bioreactor Design and Flow Conditions on Proliferation and Differentiation. In: Eberli D (Ed.). Cells and Biomaterials in Regenerative Medicine: InTech; 2014.
CrossRef
25. Rojewski MT, Fekete N, Baila S et al. GMP-compliant isolation and expansion of bone marrow-derived MSCs in the closed, automated device quantum cell expansion system. Cell Transplant. 2013; 22(11): 1981–2000.
CrossRef
26. Thomas RJ, Anderson D, Chandra A et al. Automated, scalable culture of human embryonic stem cells in feeder-free conditions. Biotechnol. Bioeng. 2009; 102(6): 1636–44.
CrossRef
27. European Commission. Good Manufacturing Practice Medicinal Products for Human and Veterinary Use. Computerised Systems. 2011; 4: Annex 11
28. Schenk FW, Kulik M, Schmitt R. Metrology-based quality and process control in automated stem cell production. Technisches. Messen. 2015; 82(6): 309–16.
CrossRef
29. Kulik M, Elanzew A, Haupt S et al. Novel platform for fully automated generation and expansion of highly standardized iPS cells. J. Biotechnol. 2016; 231(Suppl.): S33–4.
CrossRef
30. Herberts CA, Kwa MS, Hermsen HP. Risk factors in the development of stem cell therapy. J. Transl. Med. 2011; 9: 29.
CrossRef
31. Johnston C, Callum J, Mohr J, Duong A, Garibaldi A, Simunovic N, Ayeni OR, Bioburden Steering Committee and Skin Working group. Disinfection of human skin allografts in tissue banking: a systematic review report. Cell Tissue Bank. 2016; 17(4): 585–92.
CrossRef
32. Bony C, Cren M, Domergue S, Toupet K, Jorgensen C, Noël D. Adipose mesenchymal stem cells isolated after manual or water-jet-assisted liposuction display similar properties. Front Immunol. 2016; 6: 655.
CrossRef
33. Rathore AS, Winkle H. Quality by design for biopharmaceuticals. Nat. Biotech. 2009; 27(1): 26–34.
CrossRef
34. Lipsitz YY, Timmins NE, Zandstra PW. Quality cell therapy manufacturing by design. Nat. Biotech. 2016; 34(4): 393–400.
CrossRef
35. Glassey J, Gernaey KV, Clemens C et al. Process analytical technology (PAT) for biopharmaceuticals. Biotechnol. J. 2011; 6(4): 369–77.
CrossRef
36. Rathore AS, Bhambure R, Ghare V. Process analytical technology (PAT) for biopharmaceutical products. Anal. Bioanal. Chem. 2010; 398(1): 137–54.
CrossRef
37. Twomey K, Nagle LC, Said A, Barry F, Ogurtsov VI. Characterisation of nanoporous gold for use in a dissolved oxygen sensing application. BioNanoScience 2015; 5(1): 55–63.
CrossRef
38. Twomey K, O’Mara P, Pulka J et al. Fabrication and characterization of a test platform integrating nanoporous structures with biochemical functionality. IEEE Sensors J. 2015; 15(8): 4329–37.
CrossRef
AFFILIATIONS
Jelena Ochs1, Frank Barry2, Robert Schmitt3& J Mary Murphy2*
1 Fraunhofer Institute for Production Technology, Aachen, Germany.
2 Regenerative Medicine Institute (REMEDI), National University of Ireland, Galway, Ireland.
3 Laboratory for Machine Tools and Production Engineering (WZL), RWTH Aachen University, Steinbachstr. 19, 52074 Aachen, Germany.
*Author for Correspondence: mary.murphy@nuigalway.ie
This work is licensed under a Creative Commons Attribution- NonCommercial – NoDerivatives 4.0 International License.